Enhancements of direct band radiative
recombination from Ge
Professor
Chee-Wee Liu's group
Graduate Institute of Photonics and
Optoelectronics, National Taiwan
University
臺灣大學光電所
劉致為教授
Due to
the high carrier mobility, strong photon
absorption, and possible integration
with Si, the indirect band gap Ge is
used for both scientific interest and
optoelectronic applications. The methods
to enhance the direct bandgap transition
include the high pumping level, the
elevated temperature, the high
concentration of n type doping, and the
biaxial tensile strain. Significant
enhancement of direct transition
fraction in both photoluminescence (PL)
and electroluminescence (EL) is
demonstrated. The physical recombination
models are developed for both direct and
indirect transitions.
Fig. 1 and
Fig. 2 show
the PL infrared emission from the n+p Ge
structure. The electrons in Γ valley and
L valley recombine with holes in the
valence band and emit infrared at the
795meV peak (direct bandgap transition)
and the 695meV peak (indirect bandgap
transition), respectively. By increasing
pumping power and temperature, the
electron fraction in Γ valley increases
and the electron Fermi level moves
upwards. The indirect and direct bandgap
transitions are also observed in EL,
measured (Fig. 3).
The spectra of indirect and direct bandgap emission can be fitted by using
the electron-hole-plasma (EHP)
recombination model and the direct
bandgap recombination model,
respectively. To have a reasonable fit,
the band tail of absorption edge in the
direct bandgap recombination model is
taken into consideration. The intensity
fraction of direct bandgap transition
increases with increasing excitation
level and reaches 39% and 22% at EL
excitation of 600mA and PL excitation of
360mW, respectively. The intensity
fractions of direct bandgap transition
are enhanced by 4 times and 1.1 times
for EL excitation from 100mA to 600mA
and PL excitation from 120mW to 360mW,
respectively. By applying biaxial
tensile strain, the tensile strain
shrinks the direct bandgap more than the
indirect bandgap. The direct bandgap
transition becomes more and more
significant (1.8X) by increasing the
biaxial tensile strain up to 0.37% (Fig.
4).
The high PL/EL pumping level, n-type
doping concentration, and high
temperature can enhance the direct
bandgap transition. The strain can be an
extra factor to enhance the direct
transition of Ge up to ~ 1.8 times with
0.37% strain. The progressive
improvement of the radiative
recombination makes it possible to have
Ge-based light emitting devices for
practical applications.
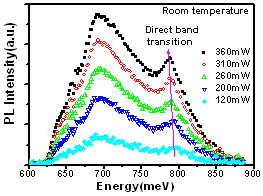 |
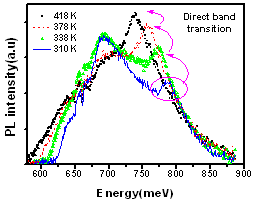 |
Fig. 1 The PL
spectra of the Ge (100) n+p diode at
room temperature. |
Fig. 2
Temperature dependent PL at the
temperature of 310 ~ 415K. |
Fig. 3 The EL
spectra of the Ge (100) n+p diode at
current (a) lower than 400mA, and
(b) higher than 400mA. |
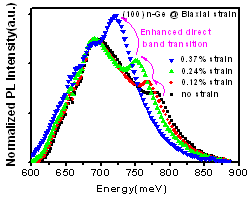 |
Fig. 4 PL
spectra of n-type bulk Ge (100)
under biaxial tensile strain. |
Efficient
and compact design of up-converted 435nm
blue lasers
Professor Lung-Han Peng's
group
Graduate Institute of Photonics and
Optoelectronics, National Taiwan
University
臺灣大學光電所 彭隆瀚教授
We
reported a design of up-conversion 435nm
blue lasers by simultaneously fulfilling
the nonlinear processes of 1st-order
quasi-phase-matching optical parametric
oscillation (QPM-OPO) with 2nd-order
second harmonic generation (QPM-SHG) in
a periodically poled lithium tantalate (PPLT)
at a single-period of 7.9μm and 75%
domain duty cycle (Fig.1). An optimum PPLT device of 15 mm length, albeit
facet uncoated, exhibits a low threshold
of 150mW and a differential slope
efficiency of 22.6%, rendering a 56mW
blue generation when pumped by a pulsed
532nm green laser with an average power
rating of 400mW (Fig.2). We noted that
the transmitted green power approached
saturated values of 108 and 200 mW at
PPLT crystal lengths of 20 and 10 mm,
respectively. This phenomenon resembles
the optical power limiting effect
earlier reported on the doubly- and
singly-resonant oscillators (DRO/SRO).
In a steady state operation, it
signifies a pinning process of the
oscillator gain at a threshold value and
suggests that excess energy from the
pump beam would be transferred to the
phase-matched nonlinear optical process.
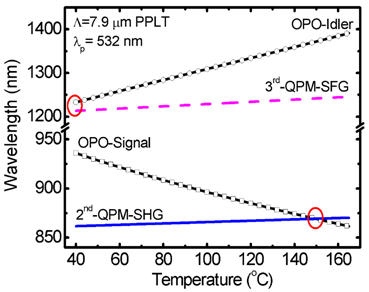 |
Fig.1:
Calculated spectral and temperature
tuning curves for the QPM-OPO and -SHG
processes in a PPLT device of 7.9
μm
period. |
Fig.2: (a)
Measurement of the up-conversion
blue efficiency of a 15 mm-long PPLT
Sample B of 7.9 μm period. (Red
dashed line represents linear
fitting to the blue laser power.)
Inset: crystal length dependence of
the differential slope efficiency
for the blue lasers measured at
400mW green pump. (b) Calculated
output power of the up-conversion
blue lasers at an optical loss
Γ of
99% and crystal length of 10, 15,
and 20mm. Inset: calculated crystal
length dependence of the
differential slope efficiency for
the blue lasers at a green pump
power of 400mW. |
Glass-Clad Crystal Fibers Based
Ultrahigh Resolution Optical Coherence
Tomography
Professor
Sheng-Lung
Huang's
group
Graduate Institute of Photonics and
Optoelectronics, National Taiwan
University
臺灣大學光電所
黃升龍教授
Optical
coherence tomography (OCT) has evolved
as a powerful diagnostic modality for
non-invasive medical applications. With
the great success of OCT in recent
years, high resolution and high image
fidelity are getting more importance.
The axial resolution is inversely
proportional to the light bandwidth. So
far, several approaches have been
proposed to achieve high axial
resolution, such as multiplexed
superluminescent diode, femtosecond
laser, continuum generation from
photonic crystal fiber, and xenon
illuminator. In general, these solutions
either have high cost or exhibit bumpy
spectrum. In contrast, spontaneous
emission light can generate broadband
and near-Gaussian spectrum, but the
power level is often very weak. Using
waveguide to collect the amplified
spontaneous emission (ASE) can improve
brightness of the source for OCT system.
A double-clad crystal fiber (DCF) growth
technique has been developed to guide
and amplify the spontaneous emission.
Using active medium as the
single-crystalline core, the DCF can
maintain the high cross section of the
crystal environment, and effectively
collect the ASE.
By
means of the laser-heated pedestal
growth (LHPG) method, single crystalline
fibers of various active media and
diameters were first obtained from
500-μm-diameter source rods with a few
diameter-reduction steps to become 30 ~
100 μm in diameters. They were then gone
through a codrawing LHPG process to form
DCFs. During the codrawing process, the
DCF cores were further reduced to 5 to
20 μm depending on applications. At
visible wavelength range, a Ce3+:YAG DCF
generating 560-nm center wavelength with
a 3-dB bandwidth of 98 nm was
fabricated. At near IR wavelength
ranges, the active media were
Ti3+:sapphire and Cr4+:YAG for a center
wavelength of 770 nm and 1380 nm,
respectively. For Ce3+:YAG DCF, the
broadband emission and short central
wavelength of this light source enable
the realization of 1.5-μm axial
resolution in air or 1.1 μm in bio
tissues. The relatively smooth spectrum
reduced the side lobe of its point
spread function, and therefore,
facilitated the generation of a high
quality image with less crosstalk
between adjacent image pixels.
As a demonstration, an
Aplocheilus Lineatus Gold fish was
adopted to map out the stroma of its
cornea in vivo as shown in Fig. 1. The
layers inside the cornea were clearly
identified. As time went on, the cornea
became thinner and more atrophic because
of water dissipation in the dry
atmosphere. This OCT system may be
useful for the detection of early stage
cornea disorders, such as Fuch’s and
keratoconus dystrophies. Active
broadband DCFs are promising for high
resolution and high image fidelity OCT
systems. Micron resolution can be
achieved with low image pixel cross
talk. These CW light sources can enable
low-cost OCT systems with cellular
resolution for various in-vivo
bio-medical applications that fulfill
the safety requirement of 20 mJ/cm2 set
by US Food and Drug Administration.
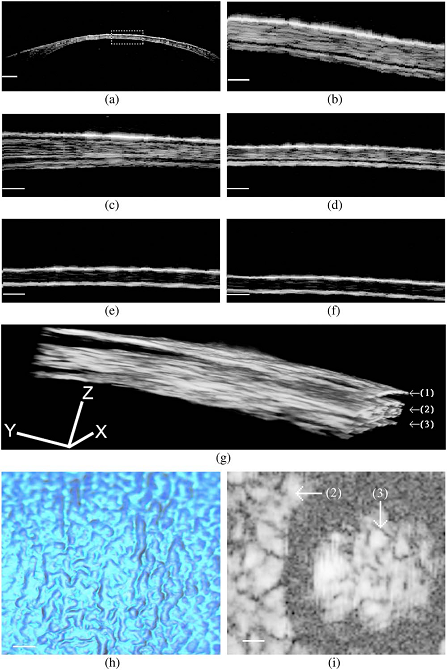 |
Fig. 1.
Goldfish cornea images of (a) full
range and (b) its partial
magnification at t=0.5 h. (b) is the
magnified figure from the dot
rectangular region of (a), where the
length of scale bar in (a) is 100 μm.
The cross-sectional image at the
same tomographic position of fish
cornea at t=0, t=0.5 h,
t=1 h, and
t=1.5 h is (c), (d), (e), and (f),
respectively. (g) is the 3D image of
oblique viewpoint at t=0. (i) is the
en-face image inside layers (2) and
(3) of (g), whereas (h) is the
cornea surface imaged by traditional
microscope. All the scale bars are
20 μm except for (a). |
Acknowledgment
The
authors would like to thank the support
from the National Taiwan University and
Foresight Taiwan Project Office,
National Science Council under grant
number NSC 98 2627-E-002-001.
|